Our System
We study the quantum collective behavior of ultracold fermionic atoms. The experiment is performed in a vacuum chamber, since it is very important to isolate the atoms from the surrounding. So, if the atoms are kept inside a vacuum chamber, how do we actually collect them, cool them, hold them in one place and manipulate them ? keep reading to find out the answer.
We are currently building our apparatus, which you can read more about here.
If you want to dive into the details of the experiment, a very good introductory paper can be downloaded here.
Cooling
The fundamental build block in our experiment is the fermionic atom (potassium 40). We collect about one billion atoms using lasers and magnetic fields. The basic idea is that we tune our laser near an atomic transition (i.e. its energy is close to the energy difference between two internal states of the atom), and use the momentum change caused by this absorption and emission to slow down the atoms. Of course, the momentum change caused by a single photon is very small, but there are many photons in a laser, and the process is actually very fast an efficient. However, at the temperature of the atoms is so low that even a scattering of a single photon heats them. At this point we turn off the lasers and move to a different cooling technique called evaporative cooling. The idea is very simple; the distribution of energies of the atoms is not symmetric. this means that there is always a chance to find atoms at a very high energy, even if the average energy of the cloud is quite small. If we remove an atom with high enough energy, we will decrease the average energy of the cloud and cool it. The trick is to be patient enough and wait until an atom with a high enough energy comes around, and then this process is efficient (we don’t need to remove many atoms in order to cool down the cloud substantially). This is why the evaporation process in ultracold atoms experiments is very slow. In the experiment, there are different techniques how to remove just the most energetic atoms. One common way to do it is to hold the atoms in a trap whose potential energy barrier can be controlled. Atoms with energy higher than the barrier are effectively not trapped and leave the trap. The evaporation process proceeds by gradually and slowly lowering this potential barrier. The time it takes to reach low enough temperatures to observe quantum effects in these experiments is typically between several seconds and up to several minutes.
The vacuum system
To reach such low temperatures, the atoms must be isolated from the surrounding. This is accomplished by keeping them in a vacuum chamber. A collision between an atom in the ensemble and an atom coming of the walls of the vacuum chamber leads to loss of the that atom. That require us to work in a very clean vacuum systems such that we minimize the probability for such an event. Since we manipulate and probe the atoms with lasers, many parts of the vacuum chamber are transparent. We also have many coils around the apparatus, which are used to control the magnetic fields. Our ultra-high vacuum system is shown in the image below. It is composed of three inter-connected chambers: a first chamber for a 2D magneto-optical trap which loads a 3D MOT in the second octagonal chamber. In the second chamber most of the cooling process is taking place, after which the atoms are transferred to the third chamber, where the real science takes place (hence this chamber is called “science chamber”).
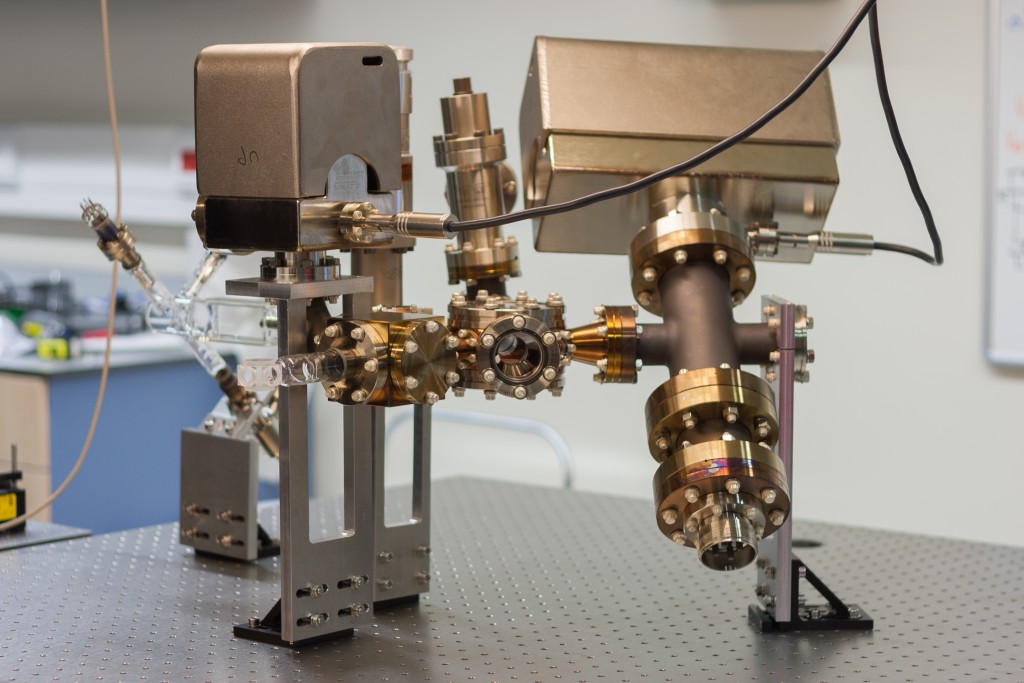
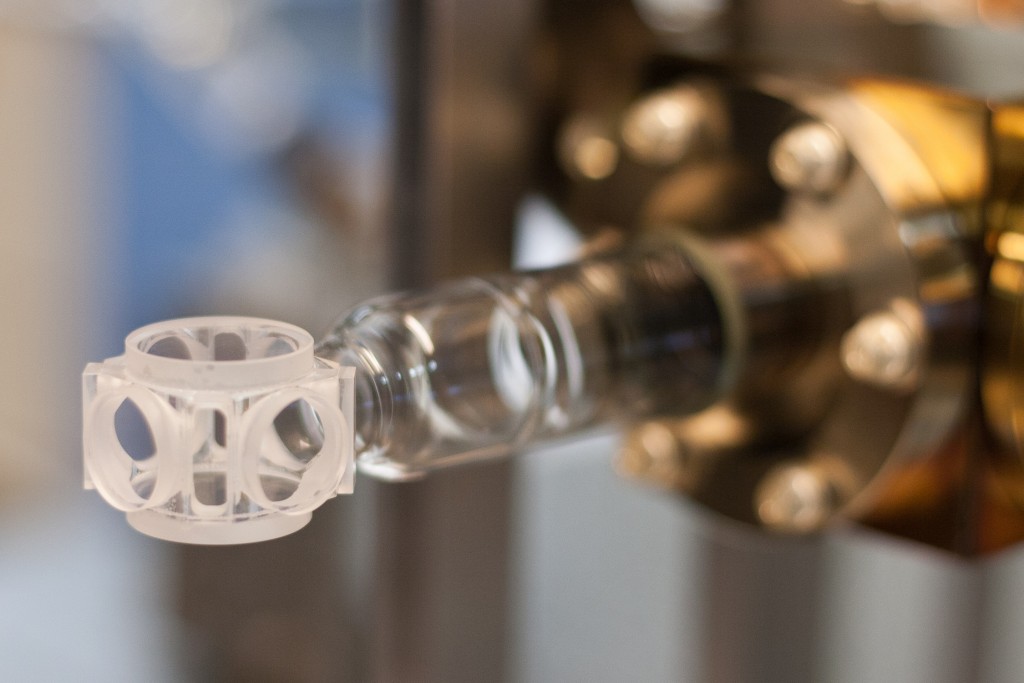
Laser systems
A crucial part in our setup is the laser system. There are lasers at many different wave lengths, which are used for cooling, manipulating the state (i.e. optical pumping), trapping the atoms (far off resonance lasers), and probing. The wavelength and intensity of all these laser need to be carefully mainlined and controlled. This is done using electro-optical devices, such as Acousto-Optical Modulators (AOM) or Electro-Optical Modulators (EOM). These devices enable us to modify the frequency, phase and intensity of the lasers. The wavelength of some of the lasers is continuously compared and locked to atomic transition lines using saturated absorption spectroscopy. When possible, we inject the lasers into optical fibers and transfer them to the vacuum chamber. The vacuum chamber, as well as the lasers and optical elements, are positioned on a special optical table that minimizes vibrations and cross-talks between different parts of the experiment.
Controlling the interaction between the atoms
One property that really sets ultracold Fermi gases apart is the ability to tune the interactions between the particles using a Fano-Feshbach resonance. For a scattering of two atoms, the resonance occurs when there is a degeneracy between the energy of the incoming free particles and that of some bound molecular state. Since the energy of the bound state depend on the magnetic field, we now have in hand a convenient knob to modify the collisional properties of the atoms. In the vicinity of the resonance the collisional properties change dramatically (this is usually quantified by the s-wave scattering length), and the effective interaction can be either attractive or repulsive. By changing the magnetic field, one can go continuously between a positive scattering length, where fermions form diatomic molecules (BEC side of the resonance), to negative scattering lengths, where they form Cooper pairs (BCS side). At resonance, the scattering length diverges and the system becomes universal (also referred to as the unitary regime). It is exactly in the BCS-BEC crossover regime that the Fermi gas shares some of the the characteristics of high-Tc superconductors, such as a pair size in the order of the mean particle separation, a high critical temperature T (in terms of the Fermi temperature), and a pseudogap normal state.